October 2023 Issue Table of Contents
Earth’s history has been punctuated by extraordinary magmatic events that produced large igneous provinces (LIPs). Many LIPs induced global changes, including millennial-scale warming, terrestrial and oceanic mass extinctions, oceanic anoxic events, and even glaciations. Research over the past 20 years has shown that shallow crustal degassing is an important factor contributing to the environmental impact of LIPs. Contact metamorphism in sedimentary basins can generate huge gas volumes, and operates as a function of magma volume and the architecture of LIP plumbing systems. Numerous open questions remain concerning the role of LIPs in triggering rapid and lasting changes, whose answers require collaboration across geoscientific disciplines. In this issue, we present the status of five key research themes and discuss potential ways forward to better understanding these large-scale phenomena.
1811-5209/23/0019-0269$2.50 DOI: 10.2138/gselements.19.5.269
Keywords: Large igneous province; sedimentary–volcanic basin; magma plumbing system; volatiles; environmental crises.
INTRODUCTION
It has been almost 20 years since large igneous provinces (LIPs) were spotlighted as a thematic issue in the first volume of Elements in 2005 (vol. 1, no. 5). Since then, the scientific literature on LIPs has proliferated. For example, the term “large igneous province” was cited in the title and/or abstract of 550 original or review articles published in 1989–2005, whereas a staggering 3400 papers were published thereafter (2006–2022), demonstrating the tremendous research efforts that have since been put into understanding the significance of LIPs in Earth history. As the articles in this issue of Elements show, our understanding of LIPs has evolved considerably over the past 20 years (see also Svensen et al. 2019). Some key areas where advances have been made involve high-precision geochronology and the role that sill emplacement plays in modulating outgassing of sedimentary rock units. Magma–host rock interaction, including crustal assimilation and contact metamorphism, has therefore become central in understanding how LIPs may have driven local and planetaryscale changes. Progress has also been made using sedimentary geochemical proxies (e.g., Hg, Ni, Te, and isotope geochemistry; Percival et al. 2018) to trace both environmental processes and the pulsed nature of LIP eruptions.
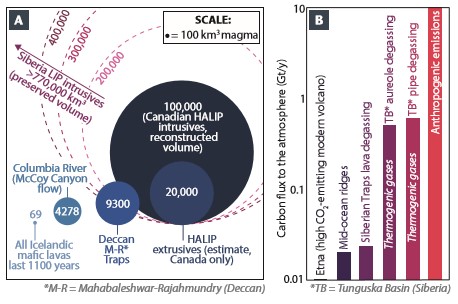
The key attributes of LIPs are widely understood to include: i) formation during anomalously productive mantle melting events with significantly higher magmatic fluxes than those associated with modern volcanic activity, ii) eruption of mostly mafic (basaltic) lavas, and iii) vertically and laterally extensive magma feeding zones (also known as magma plumbing systems) characterized by the emplacement of enormous amounts of mafic magma into the lithosphere as sheet intrusions (sills and dikes; e.g., Black et al. 2021; Mittal et al. 2021). Important to note for this issue of Elements is that many continental LIPs have plumbing systems that developed within sedimentary basins, which together can be termed sedimentary–volcanic basins, or simply volcanic basins. These include the Central Atlantic Magmatic Province (CAMP), the High Arctic LIP (HALIP), the Karoo LIP, the North Atlantic Igneous Province (NAIP), and the Siberian Traps. Seismic profiles from offshore areas have shed light on both the structure of these volcanic basins and the fate of gases generated when sedimentary rocks are heated around sills and dikes (Planke et al. 2005). In turn, field and petrological studies have shown how contact metamorphism in volcanic basins can trigger the massive production of metamorphic (i.e., thermogenic) gases that could have amplified the environmental impacts of LIPs (e.g., Svensen et al. 2009; Bédard et al. 2023).
Earth’s past is dotted with LIP events (e.g., Kasbohm et al. 2021), which helped to shape its tectonic evolution, the location of valuable resources such as metallic ores, and the history of life. This is partly because LIP events facilitated large-scale redistribution of energy and mass from the Earth’s mantle to the lithosphere, surface, and atmosphere (conversely, LIPs can also act as volatile sinks through weathering processes, as discussed below). Because of the unusually high melt production rate of LIPs and the fact that magma batches were emplaced in pulses, the bulk of the mass transfer took place over geologically short periods of time. Significant carbon degassing episodes may have, therefore, taken place on timescales as short as centuries and millennia, making LIP-related events relevant for understanding changes during the “Great Acceleration” and the Anthropocene. For these reasons, LIP research continues to attract a wide diversity of scientists, including igneous petrologists, organic and inorganic geochemists, geodynamicists, Earth system modelers, climate scientists, economic geologists, and more. The study of LIPs is thus fertile ground for inter- and trans-disciplinary research approaches and has consequently fostered the development of a vibrant and ever-growing research community. The 2021 IODP expedition 396 offshore Norway, targeted to further understand the relationships between the NAIP and the Paleocene–Eocene thermal maximum, is a recent example of the enormous scale of resources and energy devoted to international LIP-related research (Planke et al. 2022).
IN THIS ISSUE
A testament to the longevity of interest in LIPs is that they have been featured in two previous issues of Elements: “Large Igneous Provinces: Origin and Environmental Consequences” in 2005 (vol. 1, no. 5) and “Catastrophic Perturbations to Earth’s Deep Carbon Cycle” in 2019 (vol. 15, no. 5). A core goal of the current issue is to explore some of the ways in which LIPs were effective drivers of global change. Here we focus on processes that contributed to both climate warming and cooling events. Part of this issue is also dedicated to looking closer at some of the deleterious impacts that LIP events have induced in the terrestrial environment. An overview of the ways that LIP activity has triggered biotic crises is provided by Grasby and Bond (2023 this issue), a review of how contact metamorphic (i.e., thermogenic) volatiles are generated when LIPs are emplaced into sedimentary basins is given by Svensen et al. (2023 this issue), and an appraisal of how LIP-induced climate warming impacted land ecosystems is provided by Galloway and Lindström (2023 this issue). Although most LIPs are associated with climate warming, this issue spotlights a case where the emplacement of a LIP has been linked to the initiation of global icehouse conditions, potentially due to CO2-drawdown as a result of the weathering of LIP basalts (Macdonald and Swanson-Hysell 2023 this issue). Finally, our understanding of the global impacts of LIPs depends critically on the rapidly evolving fields of sedimentary proxies (covered by several of the articles in this issue) and high-precision geochronology, as reviewed by Gaynor et al. (2023 this issue).
THE SCALE OF LIP MAGMATISM
Before discussing the intrusive components of LIPs, we will first attempt to put the scale of LIP magmatism into perspective. It is tempting to compare LIPs to present-day or historical volcanism, but in many respects, LIPs overshadow all other mafic volcanoes on the planet (Fig. 1). The closest historical analogue we have for outpourings of flood basalts by LIPs is the 1783–1784 Laki eruption in Iceland, which emitted ~15 km3 of magma within eight months and is the only large-volume basaltic flood lava eruption witnessed by humans (Thordarson and Larsen 2007). Notably, these authors estimate the entire volume of basaltic lava emitted in Iceland over the past 1100 years to be ~69 km3, which is a relatively small amount of magma compared with LIP eruptions. For instance, the Mahabaleshwar-Rajahmundry Traps in India, a single eruptive unit of the Deccan LIP, is thought to represent ~9300 km3 of magma (Bryan et al. 2010). Individual eruptive units at LIPs are, in turn, dwarfed by their intrusive components, as illustrated by the Canadian portion of the High Arctic LIP, which has an estimated total intrusive magma volume of ~100,000 km3 (reconstructed by Saumur et al. 2016; note that this volume estimate does not include any of the other circum-Arctic portions of the HALIP, such as Svalbard, the Barents Sea, or Greenland). Even more extreme is the Siberian LIP, whose present-day volume of sills, lavas, and pyroclastic deposits exceeds 1,700,000 km3 (Vasiliev et al. 2000; Fig. 1A). Estimating magma volumes and rates of emplacement of LIPs is difficult, but studies of lava piles and volcanic basins suggest that the cumulative total amount of magma generated by individual LIPs exceeded 1 Mkm3 during their main phases of activity. These peaks of magmatism typically lasted up to ca. 500 ky and comprised several intensive episodes or pulses lasting around 0.1–10 ky each. Relatively small-volume precursor and/or waning stage magmatism in many cases also occurred within 1–2 My of the main phase of activity. We emphasize here that LIPs are extraordinary not only in terms of their magma volumes and production rates, but with respect to their high rates of outgassing too (Fig. 1B).
LIP PLUMBING SYSTEMS
Humans have never witnessed a LIP eruption. Therefore, to understand them, we have to rely on the rock record (e.g., outcropping lavas, sills and dikes exposed through erosion, sills intersected by drill cores, sedimentary rock successions) and geochemical and geophysical models, such as seismic images of rifted margins (Fig. 2). Our current view of how LIPs are assembled is that they consist of multiple, rapidly emplaced, large-volume eruptions each on the order of 102 to 103 km3 of magma (Fig. 2A, 2B; Bryan et al. 2010). However, these outpourings of lavas are only the tip of the iceberg. The intrusive parts of LIPs are envisaged as swarms of sills and dikes that span the entire crustal column. Uncertainties surround the magma volumes generated by LIPs because the extent of LIP intrusive components are not always easy to assess, and extrusive components are frequently heavily eroded, especially where LIPs developed in the tropics. In many cases, intrusions are only poorly exposed (if at all) or covered by thick vegetation. In the case of LIPs emplaced into oceanic crust, their partial subduction can also hinder volume estimates, such as for the Wrangellia LIP or the Greater Ontong Java Plateau. Studying the plumbing systems of LIPs is therefore challenging and often necessitates the use of industry drill cores and geophysical methods, particularly seismic reflection data. Industry data and samples have made it possible to understand the formation and evolution of partly buried provinces such as the CAMP, the Siberian Traps, and the NAIP (e.g., Planke et al. 2005; Marzoli et al., 2018; Callegaro et al. 2021). Some LIPs, however, exhibit exceptional exposure and preservation of their plumbing systems, such as the Franklin LIP on Victoria Island (Canada), the HALIP in the Canadian Arctic Islands, or the Karoo LIP in South Africa (e.g., Bédard et al. 2012, 2023; Saumur et al. 2016; Fig. 2C, 2D). In cases like these, the sill network and dike swarms that supplied melts to the surface can be observed in situ. Importantly, sills in volcanic basins commonly exhibit well-developed contact aureoles. These aureoles testify to throughflow of magma and interaction with the surrounding sedimentary host rocks during magma emplacement and cooling. Contact metamorphism of basin sediments and host rocks and the generation of thermogenic volatiles have therefore become fundamental aspects of relatively recent hypotheses linking LIPs with environmental changes (e.g., Svensen et al. 2004; Ganino and Arndt 2009; Fig. 2E).
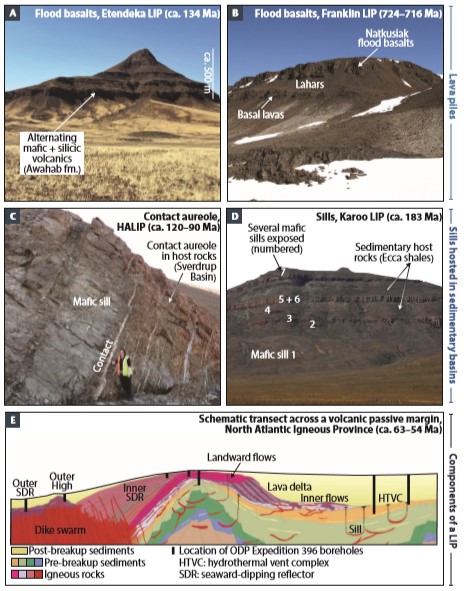
WHAT MAKES LIPS SUCH POTENT DRIVERS OF GLOBAL CHANGE?
The question “what makes LIPs such potent drivers of global change” is one that may not have a unique answer, but it motivates much of current LIP research. While a convincing temporal link exists between LIPs and environmental changes, there is apparently no single LIP feature that controls their impacts (see Kasbohm et al. 2021 for discussion). Each LIP formed differently and had the potential to induce various types of change, as the articles in this issue explore. Extrinsic conditions were perhaps more crucial than intrinsic features in determining the impacts of any given LIP. For instance, the pre-existing climate state would have been critically important, as well as the location of the LIP when it developed (e.g., tropical or high latitudes), the prevailing paleogeography (i.e., supercontinent or disaggregated land masses), the extent and efficacy of weathering, the host rocks into which the LIP was emplaced, the magma flux, the potential generation of thermogenic gases, and the ability of these gases to escape to the atmosphere and/or ocean.
The amount and type of gases released by LIPs has long been thought to play a role in the effects that LIPs had on the environment. The sheer scale of LIPs means that they would have emitted vast amounts of mantle-derived carbon and sulfur volatiles. Consider the estimates by Svensen et al. (2009) for the Siberian LIP: the amount of CO2 released from degassing of Siberian Traps flood lavas is thought to have been ca. 20,000 Gt CO2, while thermogenic volatile production from the Tunguska Basin into which Siberian sills were injected may have reached 114,000 Gt of equiva lent CO2 (Fig. 1B). This example demonstrates the reason behind the explosion of research concerning thermogenic volatiles in the past 20 years. But what are thermogenic volatiles exactly? In brief, they are gases generated when sills and dikes are emplaced into hydrocarbon accumulations or sedimentary host rocks, such as shales, carbonates, or evaporites. When host rocks are heated by magmatic intrusions, volatiles such as carbon-bearing gases (e.g., CO, CO2, CH4) are released through low- to high-temperature contact metamorphic reactions, as well as through short- timescale, high-temperature magma–sediment interactions that can occur during crustal assimilation (Deegan et al. 2022; Svensen et al. 2023 this issue; Fig. 3). These volatiles may then migrate along faults, dikes, or vent structures throughout the crust and eventually reach the atmosphere or ocean (Fig. 2E). In this way, LIPs can mobilize volatile elements that were previously locked up in sedimentary rocks and redistribute them to the surface of the planet on very short timescales.
The composition of host rocks traversed by LIP plumbing systems is crucial in determining the type of thermogenic volatiles that were generated. For instance, sedimentary basins that contain carbonaceous rocks, such as coal or shale, would have generated various types of carbon volatiles and toxic metals due to devolatilization of organic material in the hosts (Fig. 3A, 3B). Likewise, sedimentary basins that contain evaporitic rocks, such as sulfates and halides, would have generated sulfur-dominated volatiles and associated halogenated compounds (Fig. 3C, 3D). The mass of volatiles released from metamorphic aureoles can be estimated from thermodynamic models and aureole petrology (e.g., Heimdal et al. 2021; Svensen et al. 2023 this issue), while geochemical studies of magmatic rocks and contact aureoles can provide information on how much crust was assimilated by the intruding sills and how volatiles were mobilized in metamorphic aureoles (e.g., Callegaro et al. 2021; Bédard et al. 2023). Furthermore, process-related information about the assimilation and devolatilization of shale in LIP mafic melt has recently been captured in high P–T experiments (Deegan et al. 2022; Fig. 3B). The effects of thermogenic volatiles on the environment range from long-term warming to short-term cooling to ozone depletion, acid rain, and ecosystem poisoning (see Grasby and Bond 2023 this issue; Galloway and Lindström 2023 this issue).
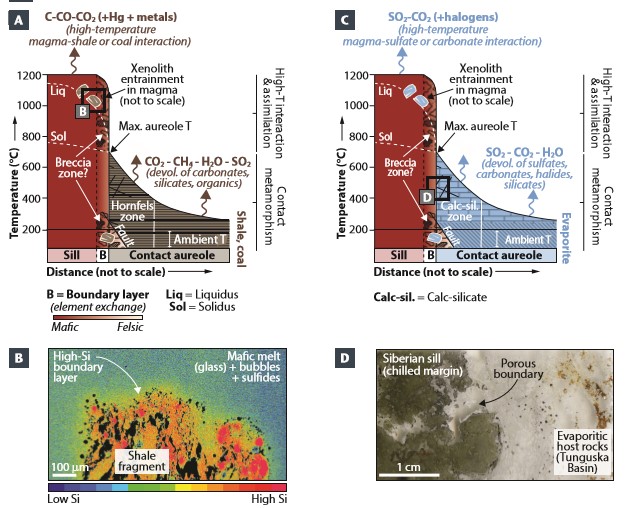
It is important to note that although thermogenic volatiles may represent a powerful factor in determining the lethality of a LIP, this hypothesis rests on the ability for thermogenic volatiles to migrate to the surface. In many cases, proof of volatile expulsion is well documented (e.g., degassing pipe structures in Siberia Traps, the Karoo LIP, and the NAIP; Svensen et al. 2009; Manton et al. 2022). However, in the case of the Canadian HALIP, it was recently suggested that a portion of the thermogenic volatiles generated during sill emplacement into the Sverdrup Basin may have been trapped in the plumbing system and, unable to escape, reacted with the surrounding rocks to produce calcite cements (see discussion in Bédard et al. 2023). Estimating gas fluxes from contact aureoles thus faces several challenges, including the construction of robust basin-scale volume estimates, accurate knowledge of the sedimentary successions at depth, and information regarding the duration and thermal history of each sill– aureole event. The take-away message is that every LIP is unique and requires careful consideration of the factors that could have helped it contribute to contemporaneous environmental crises. These factors range from the production efficacy of thermogenic volatiles to igneous gas fluxes and LIP weatherability, to coincidence with external factors such as bolide impacts.
TIMING IS EVERYTHING
A challenge with linking LIPs and environmental changes is that evidence for each typically occurs in different areas of the planet. For example, the Siberian Traps erupted in present-day northeastern Russia, but the environmental disturbance associated with this event occurred worldwide, and is recorded in sedimentary rocks as far away as Canada and Australia (e.g., Dal Corso et al. 2022). The best way to verify the LIP-environment connection is to precisely determine the timing of each and demonstrate that they were truly synchronous. Recently, there has been great progress in generating high-resolution proxy reference sections, and in combining traditional carbon isotope analyses with new proxies that record the occurrence of volcanism, such as mercury concentrations normalized to the organic carbon content. These provide a promising tool to correlate events over great distances (see Galloway and Lindström 2023 this issue; Grasby and Bond 2023 this issue; Svensen et al. 2023 this issue). The smoking gun proof of correlation is now considered to be a combination of proxy data and highly precise and accurate radioisotopic ages obtained using either the U-Pb system in zircon or the 40Ar/39Ar system in plagioclase. Increasing numbers of studies utilize the U-Pb technique in zircon, retrieving ages from either sills or dikes (see Macdonald and Swanson-Hysell 2023 this issue; Gaynor et al. 2023 this issue), whereas the most common technique for generating ages from lava flows is 40Ar/39Ar dating of plagioclase or groundmass (e.g., Marzoli et al. 2018; Antoine et al. 2022).
Also possible to date are volcanic ash horizons that bracket mass extinction events or major environmental disturbances recorded in the stratigraphic record (e.g., Schoene et al. 2010). Overall, the temporal correlation between LIP emplacement and major climatic disturbance in the Phanerozoic is well established (Kasbohm et al. 2021). However, proof of correlation brings with it more nuanced questions such as:
- Which processes during LIP emplacement are the main drivers of environmental crises?
- How important is the emplacement rate for the environmental impact?
- Are LIPs emplaced continuously—or are stop-start (stochastic) processes important?
The best way to respond to these questions is to precisely determine the timeframe for the emplacement of all the individual building blocks of a LIP and compare this chronology with the timing of changes recorded in the stratigraphic record. However, the questions above will remain outstanding until further accurate and precise dates become available.
In this issue, we outline the current understanding of the timescales of LIP events, what these ages mean, and what is missing (see Gaynor et al. 2023 this issue). It is important to recognize that most of the obtained ages for Phanerozoic LIPs are derived from shallow-level intrusions, rather than from flood basalts or deeper crustal intrusions, which could potentially bias our understanding of the timescales of LIP emplacement. For instance, high-precision dates are available for the ancient Neoproterozoic Franklin LIP, which have proven crucial for determining the relationship between its emplacement and the onset of the Sturtian Snowball Earth event (Macdonald and Swanson-Hysell 2023 this issue). However, there are still many LIP events with sparse or no high-quality age constraints, potentially leading to gaps in our understanding of the environmental impacts induced by LIPs through time (e.g., the Wrangellia LIP). We also stress here that even LIPs that are considered to be well-dated (e.g., the Siberian LIP) contain, at best, a few tens of dated samples over magma volumes on the order of a million km3 (Kasbohm et al. 2021). High-precision geochronology is costly and time-consuming; nevertheless, future endeavors in obtaining robust age constraints will undoubtedly push forward our knowledge of the timing and tempo of LIP emplacements.
FURTHER READING
Research surrounding LIPs, their magma compositions, plumbing systems, geochronology, links to environmental disasters, and fingerprints in sedimentary records, is currently flourishing. New approaches using state-of-theart geochemical modeling and experimental approaches, high-precision geochronology, and paleontological and geophysical methods (to name a few) are paving the way for exciting new discoveries. This is great news for LIP-enthusiasts, but it means that we cannot possibly cover all aspects of modern LIP research in one issue of Elements for space reasons. A small selection of additional research themes is briefly summarized below.
- The origins of LIPs may be varied (e.g., mantle plumes, melting of fertile mantle lithologies) and remain debated. Geochemistry is a widely used tool for helping to unravel LIP origins and petrogenesis. For an introduction to how trace elements can be used to “fingerprint” LIP provenance, the reader is directed to Pearce et al. (2021).
- LIPs are well-known for their association with magmatic sulfide deposits, the genesis of which in many cases required the availability of crustal sulfur. For an appraisal of how magmatic sulfides are formed and emplaced in LIPs, see Lesher (2019).
- This issue of Elements focuses on continental LIPs, but there are many examples of LIPs emplaced in oceanic crust, including the most voluminous LIP known: the Greater Ontong Java Plateau. The effects of oceanic LIPs on the environment are still uncertain, but for those wishing to explore this topic through the lens of sediment geochemistry, a good overview is provided by Percival et al. (2018).
- While the shallow parts of LIP plumbing systems are the focus of this issue, relatively little is known about their deep crustal structures (i.e. lower crustal bodies). Geophysical studies have been instrumental in illuminating this region, as shown by Abdelmalak et al. (2017).
- The sources of LIPs from the lowermost mantle are enigmatic, but what is certain is that LIPs play an important role in linking the Earth’s interior to its surface. A review of lower-mantle LIP sources (i.e., large low shear velocity provinces) and their connections to the planet’s crust and atmosphere can be found in Torsvik et al. (2021).
- The Perspective by Lee (2023 this issue) further explores the connections between the solid Earth and the atmosphere, and how interactions between the two have affected the habitability and evolution of life on Earth.
- Finally, LIPs represent planetary phenomena that are not restricted to Earth; for example, massive outpourings of mafic lava on Mars and Venus may be analogous to terrestrial LIPs. For readers interested in magmatism in the inner Solar System, a good starting point is the review by Byrne (2000).
ACKNOWLEDGMENTS
We are grateful to all the authors, reviewers, and the Editorial Team at Elements for making this issue both possible and a pleasure to be part of. We also thank R Lange, E Posner, C-T Lee, and JH Bédard for providing helpful comments that improved the text. We are especially grateful to A Marzoli for generously sharing his deep knowledge on LIPs and to JH Bédard for sharing his expertise on the HALIP. FMD acknowledges funding from Swedish Research Council (Vetenskapsrådet grant #2022-04569), SC and HHS from the Research Council of Norway (Young Research Talent grant #301096; SFF Centre for Earth Evolution and Dynamics (CEED) grant #223272), and JHFLD from NSERC and FRQNT grants.
REFERENCES
Abdelmalak MM and 6 coauthors (2017) The T-reflection and the deep crustal structure of the Vøring Margin offshore mid-Norway. Tectonics 36: 2497-2523, doi: 10.1002/2017TC004617
Antoine C and 5 coauthors (2022) 40Ar/39Ar geochronology of the Drakensberg continental flood basalts: understanding large argon isotopic variations in mafic groundmass and plagioclase size fractions. Chemical Geology 610: 121086, doi: 10.1016/j.chemgeo.2022.121086
Bédard JH and 11 coauthors (2012) Faultmediated ascent in a Neoproterozoic continental flood basalt province, the Franklin sills, Victoria Island, Canada. Geological Society of America Bulletin 124: 723-736, doi: 10.1130/B30450.1
Bédard JH and 9 coauthors (2023) Basaltic sills emplaced into organic-rich sedimentary rocks: consequences for organic matter maturation and Cretaceous paleoclimate. Geological Society of America Bulletin, doi: 10.1130/B36982.1
Black BA, Karlstrom L, Mather TA (2021) The life cycle of large igneous provinces. Nature Reviews Earth & Environment 2: 840-857, doi: 10.1038/s43017-021-00221-4
Bryan SE and 7 coauthors (2010) The largest volcanic eruptions on Earth. Earth-Science Reviews 102: 207-229, doi: 10.1016/j. earscirev.2010.07.001
Byrne PK (2020) A comparison of inner Solar System volcanism. Nature Astronomy 4: 321-327, doi: 10.1038/s41550-019-0944-3
Callegaro S and 9 coauthors (2021) Geochemistry of deep Tunguska Basin sills, Siberian Traps: correlations and potential implications for the end-Permian environmental crisis. Contributions to Mineralogy and Petrology 176: 49, doi: 10.1007/ s00410-021-01807-3
Dal Corso J and 8 coauthors (2022) Environmental crises at the Permian– Triassic extinction. Nature Reviews Earth and Environment 3: 197-214, doi: 10.1038/ s43017-021-00259-4
Deegan FM and 12 coauthors (2022) Magma–shale interaction in large igneous provinces: implications for climate warming and sulfide genesis. Journal of Petrology 63: egac094, doi: 10.1093/ petrology/egac094
Galloway JM, Lindström S (2023) Impacts of large-scale magmatism on land plant ecosystems. Elements 19: 289-295
Ganino C, Arndt NT (2009) Climate changes caused by degassing of sediments during the emplacement of large igneous provinces. Geology 37: 323-326, doi: 10.1130/G25325A.1
Gaynor SP, Davies HLF, Schaltegger (2023) High-precision geochronology of LIP intrusions: records of magma–sediment interaction. Elements 19: 302-308
Grasby SE, Bond DPG (2023) How large igneous provinces have killed most life on Earth—numerous times. Elements 19: 276-281
Heimdal TH, Goddéris Y, Jones MT, Svensen HH (2021) Assessing the importance of thermogenic degassing from the Karoo Large Igneous Province (LIP) in driving Toarcian carbon cycle perturbations. Nature Communications 12: 6221, doi: 10.1038/s41467-021-26467-6
Kasbohm J, Schoene B, Burgess S (2021) Radiometric constraints on the timing, tempo, and effects of large igneous province emplacement. In: Ernst RE, Dickson AJ, Bekker A (eds) Large Igneous Provinces: A Driver of Global Environmental and Biotic Changes, Volume 255 (First Edition). American Geophysical Union, John Wiley and Sons Inc, Washington DC, pp 27-82, doi: 10.1002/9781119507444.ch2
Lee C-TA, Jiang H, Dasgupta R, Torres M (2019) A framework for understanding whole-Earth carbon cycling. In: Orcutt BN, Daniel I, Dasgupta R (eds) Deep Carbon: Past to Present. Cambridge University Press, Cambridge, pp 313-357, doi: 10.1017/9781108677950
Lesher CM (2019) Up, down, or sideways: emplacement of magmatic Fe–Ni–Cu–PGE sulfide melts in large igneous provinces. Canadian Journal of Earth Sciences 56: 756-773, doi:10.1139/cjes-2018-0177
Macdonald FA, Swanson-Hysell NL (2023) The Franklin Large Igneous Province and Snowball Earth initiation. Elements 19: 296-301
Manton B and 9 coauthors (2022) Characterizing ancient and modern hydrothermal venting systems. Marine Geology 447: 106781, doi: 10.1016/j. margeo.2022.106781
Marzoli A and 9 coauthors (2018) The Central Atlantic Magmatic Province (CAMP): a review. In: Tanner L (ed) The Late Triassic World, Topics in Geobiology, Volume 46. Springer, Cham, pp 91-125, doi: 10.1007/978-3-319-68009-5_4
Mittal T, Richards MA, Fendley IM (2021) The magmatic architecture of continental flood basalts I: observations from the Deccan Traps. Journal of Geophysical Research: Solid Earth 126: e2021JB021808, doi: 10.1029/2021JB021807
Pearce JA, Ernst RE, Peate DW, Rogers C (2021) LIP printing: use of immobile element proxies to characterize large igneous provinces in the geologic record. Lithos 392-393: 106068, doi: 10.1016/j. lithos.2021.106068
Percival LME and 10 coauthors (2018). Does large igneous province volcanism always perturb the mercury cycle? Comparing the records of Oceanic Anoxic Event 2 and the end-Cretaceous to other Mesozoic events. American Journal of Science 318: 799-860, doi: 10.2475/08.2018.01
Planke S, Rasmussen T, Rey SS, Myklebust R (2005) Seismic characteristics and distribution of volcanic intrusions and hydrothermal vent complexes in the Vøring and Møre basins. In: Doré AG, Vining BA (eds) Petroleum Geology: North-West Europe and Global Perspectives – Proceedings of the 6th Petroleum Geology Conference, Volume 6. Geological Society, London, pp 833-844, doi: 10.1144/0060833
Planke S, Berndt C, Alvarez Zarikian CA, the Expedition 396 Scientists (2022). Expedition 396 preliminary report: mid-Norwegian continental margin magmatism and paleoclimate implications. International Ocean Discovery Program, doi: 10.14379/iodp.pr.396.2022
Saumur BM, Dewing K, Williamson M-C (2016) Architecture of the Canadian portion of the High Arctic large igneous province and implications for magmatic Ni–Cu potential. Canadian Journal of Earth Sciences 53: 528-542, doi: 10.1139/ cjes-2015-0220
Schoene B, Guex J, Bartolini A, Schaltegger U, Blackburn TJ (2010) Correlating the end-Triassic mass extinction and flood basalt volcanism at the 100 ka level. Geology 38: 387-390, doi: 10.1130/ G30683.1
Svensen H and 6 coauthors (2004) Release of methane from a volcanic basin as a mechanism for initial Eocene global warming. Nature 429: 542-545, doi: 10.1038/ nature02566
Svensen H and 6 coauthors (2009) Siberian gas venting and the end-Permian environmental crisis. Earth and Planetary Science Letters 277: 490-500, doi: 10.1016/j. epsl.2008.11.015
Svensen HH and 6 coauthors (2019) Thinking about LIPs: a brief history of ideas in large igneous province research. Tectonophysics 760: 229-251, doi: 10.1016/j.tecto.2018.12.008
Svensen HH, Jones MT, Mather TA (2023) Large igneous provinces and the release of thermogenic volatiles from sedimentary basins. Elements 19: 282-288
Thordarson T, Larsen G (2007) Volcanism in Iceland in historical time: volcano types, eruption styles and eruptive history. Journal of Geodynamics 43: 118-152, doi: 10.1016/j.jog.2006.09.005
Torsvik TH and 6 coauthors (2021) Connecting the deep Earth and the atmosphere. In: Marquardt H, Ballmer M, Cottaar S, Konter J (eds) Mantle Convection and Surface Expressions, Geophysical Monograph Series, Volume 263. American Geophysical Union, Washington DC, pp 413-453, doi: 10.1002/9781119528609.ch16
Vasil’ev YR, Zolotukhin VV, Feoktistov GD, Prusskaya SN (2000) Evaluation of the volume and genesis of Permo-Triassic trap magmatism on the Siberian Platform. Russian Geology and Geophysics 41: 1696-1705 (in Russian)
October 2023 Issue Table of Contents
Large igneous provinces (LIPs) can potentially cause cooling on tens- to thousand-year timescales via injection of sulfur aerosols to the troposphere, and on million-year timescales due to the increase of global weatherability. The ca. 719-Ma Franklin LIP preceded onset of the Sturtian Snowball Earth glaciation by less than two million years, consistent with CO2 drawdown due to weathering of Ca- and Mg-rich LIP basalts, which may have contributed to cooling past a critical runaway ice-albedo threshold. A relatively cool background climate state and Franklin LIP emplacement near a continental margin in the warm wet tropics may have been critical factors for pushing the Earth’s climate past the threshold of runaway glaciation.
1811-5209/23/0019-0296$2.50 DOI: 10.2138/gselements.19.5.296
Keywords: Large igneous provinces; Franklin LIP; Sturtian glaciation; Snowball Earth; weatherability; sulfur aerosols; CO2.
INTRODUCTION
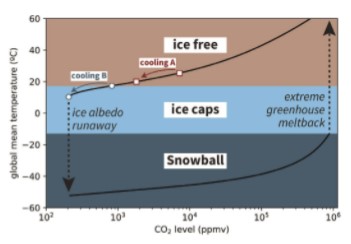
Snowball Earth glaciations are initiated once a critical threshold is reached for runaway ice-albedo feedback, where the growth of ice-sheets, which reflect rather than absorb solar radiation relative to land and ocean, leads to additional cooling and equatorward expansion of ice until it covers the globe (Pierrehumbert et al. 2011; Walker et al. 1981). Following an ice-albedo runaway, Earth can remain globally glaciated over a wide range of CO2 levels, resulting in a long-lived Snowball climate. This bifurcation of Earth’s climate system, where it can either be ice-free or in a Snowball at a given CO2 level, is a persistent feature of climate models of varying complexity (Fig. 1). Both the entrance to the Snowball state through ice-albedo runaway and exit through extreme greenhouse meltback are threshold problems in which the background paleogeography and climate sets the sensitivity to CO2 or albedo perturbations that can push the Earth to a new climate state (Fig. 1).
The geological record preserves evidence of the Snowball Earth climate bifurcation. Cryogenian (720–635 Ma) sedimentary rocks deposited under the influence of ice are present on every continent. Paleomagnetic data reveal that ice extended to the tropics twice during the Cryogenian Period and persisted for millions of years (Macdonald et al. 2010). Yet, it remains unknown what factors led to initiation of these Snowball Earth glaciations. A close temporal relationship between emplacement of the 719-Ma Franklin LIP (Fig. 2) and onset of the first Cryogenian Snowball Earth, the Sturtian glaciation, has suggested causal links (Macdonald et al. 2010; Macdonald and Wordsworth 2017; Pu et al. 2022; Dufour et al. 2023). Although LIPs are more commonly portrayed as drivers of global warming through the release of CO2, LIPs could also drive cooling towards a critical threshold due to two potential mechanisms: an albedo perturbation through eruption of sulfur aerosols (Macdonald and Wordsworth 2017), or an increase in global weatherability that draws down CO2 through increased efficiency of silicate weathering (Macdonald et al. 2010; Pu et al. 2022). The term “weatherability” refers to the cumulative factors that affect chemical weathering and associated CO2 sequestration aside from climate change, such as lithology, topography, and latitudinal distribution of continents and orogens. Continental flood basalts associated with LIPs have been hypothesized as an efficient sink for CO2, thereby increasing weatherability. Due to the relatively high concentrations of Ca and Mg in these rocks, they can sequester more carbon through carbonate precipitation in the ocean than typical crustal compositions (Dessert et al. 2001; Park et al. 2020b). Key to evaluating these potential mechanisms for cooling past a critical threshold are high-precision geochronological and paleomagnetic constraints, as both the albedo and weathering hypotheses make predictions for the timing of the Franklin LIP relative to onset of the Sturtian glaciation, and both albedo and chemical weathering are strongly dependent on latitude.
FRANKLIN LIP MAGMATISM
Lavas, sills, and dikes associated with the Franklin LIP span the Arctic margin of North America from the Yukon of NW Canada to the NW margin of Greenland (Fig. 2). The dikes form a radiating pattern and presumably fed large flood basalts across the region that have since been largely eroded, although the original surface extent is inherently uncertain. In a compilation of LIPs, Ernst et al. (2021) used the preserved record of intrusive rocks to estimate a Franklin LIP area of 2.6 Mkm2 (Fig. 2). If a polygon is extended to include additional recently identified Franklin LIP occurrences, the area increases to 3.1 Mkm2 (Fig. 2). Extending the area across the Arctic continental shelf to the inferred position of the plume head from radiating dikes leads to an area of 4.5 Mkm2, which would be larger still if the area further extended onto the interpreted conjugate continent of Siberia, as interpreted by Ernst et al. (2023). This area estimate exceeds the 3.5 Mkm2 estimate of the Siberian Traps, with the only LIPs interpreted to exceed the area of the Franklin LIP being the Central Atlantic Magmatic Province (CAMP) and the High Arctic LIP.
Preserved extrusive volcanic rocks directly associated with the Franklin LIP include the Natkusiak Formation basalt of the Minto Inlier, Victoria Island (Jefferson et al. 1985; Williamson et al. 2016) and the Kikiktat volcanics of Arctic Alaska (Cox et al. 2015). Other potential occurrences of Franklin LIP magmatism include rift-related rocks of the Mount Harper Volcanics in the Yukon and the Tatonduk Suite along the Yukon–Alaska border (Cox et al. 2018; Macdonald et al. 2018), and the Irkutsk Magmatic Province of Siberia (Ernst et al. 2023).
Natkusiak Formation, Victoria Island
The best exposures of the intrusive and extrusive relationships of Franklin LIP units are on Victoria Island, where the Shaler Supergroup culminates with the Natkusiak Formation (Jefferson et al. 1985). To the northeast, thinning of units below the Natkusiak Formation has been interpreted to record doming of the crust associated with a mantle plume (Rainbird 1993). The Natkusiak basalts are ~1100 m thick in northern exposures of the inlier (Jefferson et al. 1985) and only ~200 m thick in the south where the middle and upper members were eroded away below the sub-Cambrian unconformity (Williamson et al. 2016).
Co-magmatic diabase sills range from 1–100 m thick and show evidence for both fractional crystallization and crustal contamination, with olivine accumulation at their base and pegmatites and felsic enclaves in their upper levels (Heaman et al. 1992). Sills eviscerate the >4-km-thick Shaler Supergroup, which includes organic-rich shale and sulfate evaporites, and display extensive geochemical evidence for assimilation (Hayes et al. 2015). The shales and evaporites were hypothesized to be a source of sulfur for outsized aerosol production during LIP magmatism (Macdonald and Wordsworth 2017).
Kikiktat Volcanics, Arctic Alaska
The Kikiktat volcanics form a 10–1000-m-thick suite of lavas on the North Slope subterrane of Arctic Alaska (Cox et al. 2015). This allochthonous block was positioned against Arctic Canada during emplacement of the Franklin LIP, but its exact position along Laurentia’s northern margin remains uncertain (Macdonald et al. 2009). The Kikiktat volcanics are overlain by a poorly-sorted volcaniclastic sandstone with a dominant zircon population dated at 719.5 ± 0.2 Ma, which is interpreted to closely approximate the age of the underlying volcanics, and provides a strong tie with the Franklin LIP (Cox et al. 2015). Although the volcaniclastic unit does not record direct evidence for glaciation, it is overlain by Sturtian glacial deposits of the Hula Hula diamictite (Macdonald et al. 2009).

Mount Harper Volcanics and Tatonduk Suite, Yukon–Alaska Border
In the Ogilvie Mountains of the Yukon, the Mount Harper volcanics consist of >1000 m of basalt with minor rhyolite near the top (Macdonald et al. 2018). Sturtian-age glacial diamictite of the Eagle Creek Formation overlies the basaltic portion of the complex, but is not in contact with the rhyolite domes. Geochronology on the rhyolite domes and volcanic tuffs interbedded with the Eagle Creek Formation provide constraints on the onset of the Sturtian glaciation discussed below. Along the Alaska–Yukon border, ~50 km west of Mount Harper, the Tatonduk Suite includes dikes and ~330 m of pillowed and massive basalt flows and volcaniclastic breccia that are overlain by glacial deposits of the Rapitan Group (Cox et al. 2018). A N–S trending sub-vertical dike dated with U-Pb TIMS on baddeleyite at 713.7 ± 0.2 Ma (weighted mean 206Pb/238U age) was used to argue that the Tatonduk Suite post-dated the Mt. Harper Volcanics and was emplaced during, not before, the Sturtian glaciation (Cox et al. 2018). However, these data have large errors in the 207Pb/235U ratio and may more conservatively be interpreted to overlap in age with the Franklin LIP with an upper intercept date of 718.7 ± 16.5 Ma.
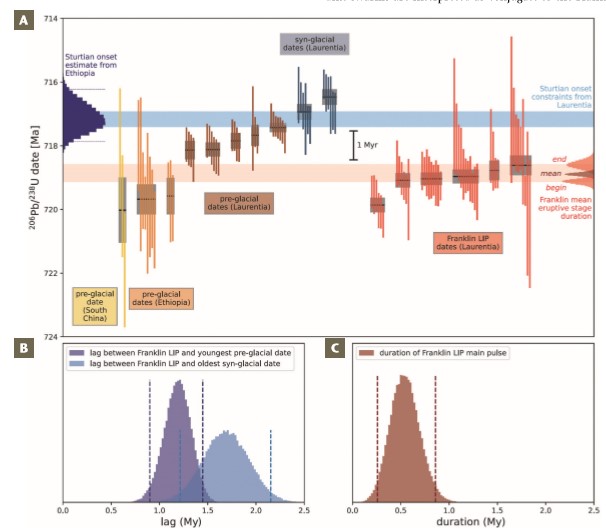
Irkutsk Magmatic Province, Siberia
Outside of North America, the Irkutsk LIP of southern Siberia has been correlated with the Franklin LIP and consists of gabbro complexes that have been dated between 730 and 710 Ma (Ernst et al. 2023). The radiating Sayan and Baikal dike swarms are interpreted as conjugate to the Franklin LIP radiating swarm in North America. Paleogeographic juxtaposition of the continents is consistent with existing paleomagnetic data, including the ca. 760-Ma Kitoi dikes paleomagnetic pole of Siberia (Evans et al. 2016).
RELATIVE TIMING OF FRANKLIN LIP AND ONSET OF STURTIAN GLACIATION
Timing of the Franklin LIP
Published U-Pb zircon and baddeleyite ages from Franklin LIP rocks span ca. 730–710 Ma (Heaman et al. 1992; Macdonald and Wordsworth 2017). However, this spread may be attributed to complications with baddeleyite geochronology that can limit both precision and accuracy (Pu et al. 2022; Dufour et al. 2023). As a result, it is preferable to base interpretations of LIP duration and the relationship with glaciation on U-Pb zircon dates developed through chemical abrasion isotope dilution-thermal ionization mass spectrometry (CA-ID-TIMS).
Two recent studies have published new CA-ID-TIMS U-Pb zircon dates that provide more precise and accurate estimates of the timing and duration of Franklin LIP magmatism in both eastern and western Arctic Canada (Pu et al. 2022; Dufour et al. 2023). These dates are all between 720 and 718 Ma (Fig. 3). This interval is defined by a first pulse of low-volume and low-Ti Type-1 plume head magmatism (date of 719.86 ± 0.21 Ma) and more voluminous Type-2 magmatism (dates of 719.08 ± 0.22 Ma, 719.04 ± 0.19 Ma, 718.96 ± 0.19 Ma, 718.77 ± 0.30 Ma, 718.61 ± 0.30 Ma, 718.61 ± 0.30 Ma, and 718.33 ± 0.18; see Beard et al. (2018) for the definitions of types 1 and 2 magmatism). These dates and their uncertainties can be used to estimate a duration of the main stage of Franklin LIP activity of 540,000 years (95% range of 260,000 to 860,000 years), lasting from ca. 719.1 to 718.6 Ma.
Timing of Sturtian Glaciation Onset
In the Yukon, glacial deposits of the Eagle Creek Formation contain interbedded volcanic tuffs dated with U-Pb CA-IDTIMS zircon between 716.9 and 716.5 Ma and underlying rhyolite domes and flows between 718.1 and 717.4 Ma (Fig. 3; Macdonald et al. 2010, 2018). These dates have been interpreted to constrain the onset age of glaciation, postdating the youngest Mount Harper volcanics date of 717.43 ± 0.14 Ma (Macdonald et al. 2010), and pre-dating the oldest syn-glacial tuff date of 716.94 ± 0.24 Ma (Macdonald et al. 2018). This ca. 717-Ma age for Sturtian glacial onset is consistent with age constraints on glacial initiation from Ethiopia (MacLennan et al. 2018) and South China (Lan et al. 2020), such that it remains the most parsimonious interpretation for the data.
The emplacement age of the main phase of the Franklin LIP from ca. 719.1 to 718.6 Ma is therefore older than the onset of Sturtian glaciation between 717.4 ± 0.2 Ma and 716.9 ± 0.4 Ma. The time lag between the end of the main pulse of Franklin volcanism and the onset of the Sturtian Snowball Earth can be estimated by comparing the main pulse end dates with the youngest pre-glacial date, resulting in an estimated lag of 1.19 My (95% confidence range of 0.90 to 1.45 My), or by comparison with the oldest syn-glacial date, with an estimated lag of 1.69 Ma (95% range of 1.21 to 2.15 Ma; Fig. 3). Overall, these constraints indicate that there was approximately 1 to 2 million years between the largest pulse of Franklin LIP magmatism and the onset of low-latitude glaciation associated with the Sturtian Snowball Earth (Fig. 3).
PALEOMAGNETISM AND PALEOLATITUDE
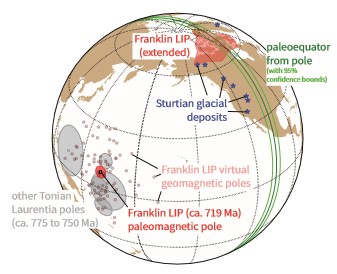
Paleomagnetic data from 83 intrusions associated with the Franklin LIP have been used to develop a mean paleomagnetic pole (Denyszyn et al. 2009), which shows that the Franklin LIP erupted in the tropics straddling the equator. On Earth today, land areas within 15° of latitude from the equator are the warmest and wettest places on Earth, and form the region known as the tropical rain belt (Park et al. 2020b). Given that enhanced precipitation in the tropics is the result of large-scale atmospheric circulation that arises on a differentially heated rotating Earth, it is reasonable to assume a similar distribution of climate belts at 719 Ma to those today. The Franklin paleomagnetic pole constrains ~95% of the LIP area to have been within 15° of the equator, and ~80% of the LIP was emplaced within 10° of the equator where it would have a high propensity to be warm and wet. Although climate sensitive sedimentary rocks indicate that the tropical rain belt was broadly associated with equatorial latitudes through the Phanerozoic, depending on regional circulation patterns, the arid belt can creep towards the equator given the high evaporative potential, which can be exacerbated in the interior of large continents. While there is evidence of arid conditions locally on Laurentia in the form of ca. 750-Ma evaporite deposits in northwestern Canada, the broad extent of the Franklin LIP within the tropical rain belt is consistent with much of it being in regions where precipitation exceeded evaporation (Fig. 4).
This Franklin LIP pole is a key constraint on Neoproterozoic paleogeography and is the youngest in a paleomagnetic pole path that comes from the ca. 777-Ma Gunbarrel LIP and the Chuar-Uinta Mountains-Pahrump basins that indicate a low-latitude position of Laurentia throughout the late Tonian Period (Fig. 4). While relatively low paleointensities of the geomagnetic field have been estimated from Franklin LIP units (Lloyd et al. 2021), the coherency of Laurentia’s pole path with a similar pole position obtained from Tonian rocks across the continent, as well as the consistency of virtual geomagnetic pole positions within the LIP itself, lend high-confidence to the pole. Together, these data are consistent with the geomagnetic field being stable and dipolar through the late Tonian (reinforced by strong paleointensity values ca. 750 Ma; Lloyd et al. 2021). In addition to constraining the Franklin LIP to have erupted in the tropics, the Franklin LIP pole also indicates that Sturtian glacial deposits were deposited near the equator (Fig. 4; Macdonald et al. 2010).
WEATHERABILITY AND SNOWBALL INITIATION
There are two hypotheses associated with the onset of ice-albedo runaway that could directly implicate the Franklin LIP: 1) a decline in CO2 levels through carbon cycle perturbations, and 2) a decrease in incoming solar radiation through the injection of sulfate aerosols into the stratosphere (Macdonald and Wordsworth 2017). The second hypothesis requires a close temporal linkage between the largest Franklin eruptions and the onset of the ice-albedo runaway that initiated Snowball Earth. The new geochronology displays a lag between the main phase of Franklin volcanism and Snowball onset, which appears inconsistent with the hypothesis that an albedo perturbation was the primary driver.
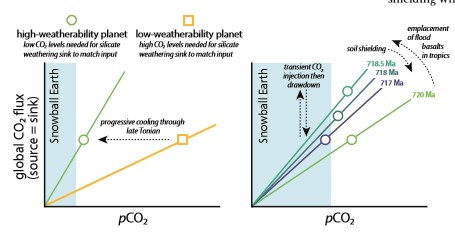
The more probable connection between the Franklin LIP and a carbon-cycle perturbation is that efficient chemical weathering of Ca- and Mg-rich basaltic rocks emplaced in the tropics may have lowered CO2 levels below the critical threshold for an ice-albedo runaway. Earth’s long-term climate is set by the sources and sinks of CO2. On millionyear timescales, the main source of CO2 is volcanism, while the main sink of CO2 is the chemical weathering of silicate rocks, ultimately leading to the formation of carbonate in the oceans. The climate dependence on chemical weathering provides a feedback that stabilizes CO2 levels and Earth’s climate state (Walker et al. 1981). In this framework, there are two principal ways to shift climate to a warmer or cooler equilibrium on geologic timescales: 1) a long-term change in CO2 outgassing rate; or 2) a change in the efficiency of CO2 sequestration through chemical weathering, that is, weatherability (Fig. 5).
Global weatherability during breakup of the supercontinent Rodinia may have been high due to an abundance of low-latitude continental margins in the tropical weathering belt. Having a concentration of land near the equator can also slightly decrease global temperature due to the increased reflectivity of land (higher albedo) relative to the sea (Eberhard et al. 2023). An additional factor associated with a low-latitude supercontinent is that as ice-sheets grow, they shield continents from both physical erosion and chemical weathering, resulting in reduced silicate weathering—a negative feedback (Marshall et al. 1988). This feedback is weakened with a low-latitude continental configuration. Weatherability can also change with distribution of high topographic relief, typically associated with active tectonics, between the warm and wet tropics and higher latitudes with less efficient weathering (Park et al. 2020a). Thus, a paleogeographic distribution of continents most sensitive to a runaway ice-albedo catastrophe is one with a maximum number of continental fragments (minimal continentality) distributed at low- and mid-latitudes, with no polar continents, and active collisions in the tropics (Eberhard et al. 2023). Geological data suggest that many of these features were achieved during the late Tonian and Cryogenian with the breakup of Rodinia followed by arc-continent collisions that likely occurred in the tropics (Park et al. 2020c). Within this paleogeographic context, enters the Franklin LIP.
Models and proxy data for pCO2 following LIP emplacement show that warming from the input of volcanic CO2 into the atmosphere will be counteracted by silicate weathering within ~1–2 My of emplacement, turning the LIP into a net CO2 sink (Dessert et al. 2001; Schaller et al. 2012). Subsequently, the development of regolith and soil shielding will mute the effects of a LIP on weatherability (Park et al. 2020b; Fig. 5). Coupled climateweathering models predict that the removal of recent flood basalt provinces on Earth today without any prescribed topographic change would change steady state pCO2 by >100 ppm (Park et al. 2020a), demonstrating the potential for cooling associated with the weatherability of LIPs.
CHALLENGES TO CAUSAL CONNECTIONS
A challenge to a causal relationship between the Franklin LIP and onset of Snowball Earth is that the second Cryogenian Snowball Earth event, the Marinoan glaciation, occurred <20 My after the end of the first Sturtian glaciation and does not appear to be associated with a LIP. This suggests that not all ice-albedo runaways are associated with LIPs and that Cryogenian paleogeographic boundary conditions were sufficient for another perturbation to initiate a second Snowball Earth.
Another challenge to the interpretation of a causal connection is that there are other similarly large LIPs emplaced at both equatorial and mid-latitudes that are not associated with glaciation, such as the CAMP and the Siberian Traps. However, both of these provinces formed when the Earth was warm and had a climate and paleogeography that was not sensitive to such a perturbation. Early-Jurassic climate records suggest cooling ~1 My after CAMP emplacement (Schaller et al. 2012), but as the weatherability increase associated with the LIP dissipated, the Earth stayed in an ice-free climate state (Fig. 5). Overall, there is a lack of correlation between LIPs and cooling trends throughout the Phanerozoic (Park et al. 2020b). Although LIP emplacement is not uniquely correlated with cooling, this result does not imply that LIPs are incapable of pushing the climate past the runaway ice-albedo catastrophe threshold in conjunction with other factors driving longer term cooling (Fig. 1).
The circumstances of the emplacement of the Franklin LIP were optimal for it to have an effect on climate. In addition to being the largest Neoproterozoic LIP preserved in the geological record and one of the largest in Earth history (Fig. 2), abundant high-quality paleomagnetic data from the province constrain it to have been emplaced in the tropics straddling the equator (Fig. 4). Emplacement in the tropical rain belt would have facilitated efficient chemical weathering, which could have been further enabled by a paleogeographic position near an open margin with tropical easterlies bringing moisture inland from the Asgaard Sea offshore of Greenland. The pulse of excess CO2 input from the LIP eruptions would have been drawn down through silicate weathering with the enhanced weatherability due to the LIP, leading to cooling beyond the initial climate state (Fig. 5). A cool climate has been suggested ~35 My prior to the onset of the Sturtian glaciation (MacLennan et al. 2020), but there are few constraints on the climate state for the intervening 35 My. If it turns out that the Earth was in a cool, partially glaciated climate state in the latest Tonian, then the Franklin may have been the LIP that broke the climate’s back.
ACKNOWLEDGMENTS
This work was supported by NSF FRES grants 1926001 and 1925990. We thank Robin Wordsworth, Dave Evans, Joshua Davies, Jean Bédard, and an anonymous reviewer for helpful suggestions. Code for the energy balance model shown in Figure 1 is available at https://doi.org/10.5281/ zenodo.10152708. Code and data for other figures are available at https://doi.org/10.5281/zenodo.10152921.
REFERENCES
Beard CD, Scoates JS, Weis D, Bédard JH, Dell’Oro TA (2018) Geochemistry and origin of the Neoproterozoic Natkusiak flood basalts and related Franklin sills, Victoria Island, Arctic Canada. Journal of Petrology 58: 2191-2220, doi: 10.1093/ petrology/egy004
Cox GM, Halverson GP, Denyszyn S, Foden J, Macdonald FA (2018) Cryogenian magmatism along the north-western margin of Laurentia: plume or rift? Precambrian Research 319: 144-157, doi: 10.1016/j. precamres.2017.09.025
Cox GM and 6 coauthors (2015) Kikiktat volcanics of Arctic Alaska—melting of harzburgitic mantle associated with the Franklin large igneous province. Lithosphere 7: 275-295, doi: 10.1130/ L435.1
Denyszyn SW, Halls HC, Davis DW, Evans DAD (2009) Paleomagnetism and U–Pb geochronology of Franklin dykes in High Arctic Canada and Greenland: a revised age and paleomagnetic pole for constraining block rotations in the Nares Strait region. Canadian Journal of Earth Sciences 46: 689-705, doi: 10.1139/E09-042
Dessert C and 6 coauthors (2001) Erosion of Deccan Traps determined by river geochemistry: impact on the global climate and the 87Sr/86Sr ratio of seawater. Earth and Planetary Science Letters 188: 459-474, doi: 10.1016/S0012-821X(01)00317-X
Dufour F and 5 coauthors (2023) New U–Pb CA-ID TIMS zircon ages implicate the Franklin LIP as the proximal trigger for the Sturtian Snowball Earth event. Earth and Planetary Science Letters 618: 118259, doi: 10.1016/j.epsl.2023.118259
Eberhard J and 5 coauthors (2023) Sensitivity of Neoproterozoic Snowball-Earth inceptions to continental configuration, orbital geometry, and volcanism. Climate of the Past 19: 2203-2235, doi: 10.5194/ cp-19-2203-2023
Ernst RE and 6 coauthors (2023) Identification of the ca. 720 Ma Irkutsk LIP and its plume centre in southern Siberia: the initiation of Laurentia–Siberia separation. Precambrian Research 394: 107111, doi: 10.1016/j.precamres.2023.107111
Ernst RE and 8 coauthors (2021) Large igneous province record through time and implications for secular environmental changes and geological time‐scale boundaries. In: Ernst RE, Dickson AJ, Bekker A (eds) Large Igneous Provinces: A Driver of Global Environmental and Biotic Changes, Volume 255 (First Edition). American Geophysical Union, John Wiley and Sons Inc, Washington DC, pp 1-26, doi: 10.1002/9781119507444.ch1
Evans DA, Veselovsky RV, Petrov PY, Shatsillo AV, Pavlov VE (2016) Paleomagnetism of Mesoproterozoic margins of the Anabar Shield: a hypothesized billion-year partnership of Siberia and northern Laurentia. Precambrian Research 281: 639-655, doi: 10.1016/j. precamres.2016.06.017
Hayes B and 6 coauthors (2015) Sulfide immiscibility induced by wall-rock assimilation in a falut-guided basaltic feeder system, Franklin large igneous province, Victoria Island (Arctic Canada). Economic Geology 110: 1697-1717, doi: 10.2113/ econgeo.110.7.1697
Heaman LM, LeCheminant AN, Rainbird RH (1992) Nature and timing of Franklin igneous events, Canada: implications for a Late Proterozoic mantle plume and the break-up of Laurentia. Earth and Planetary Science Letters 109: 117-131, doi: 10.1016/0012-821X(92)90078-A
Jefferson C, Nelson W, Kirkham R, Reedman J, Scoates R (1985) Geology and copper occurrences of the Natkusiak basalts, Victoria Island, District of Franklin. Geological Survey of Canada Current Research 85-1A: 203-214, doi: 10.4095/120045
Lan Z and 6 coauthors (2020) Toward refining the onset age of Sturtian glaciation in South China. Precambrian Research 338: 105555, doi: 10.1016/j. precamres.2019.105555
Lloyd SJ, Biggin AJ, Halls H, Hill MJ (2021) First palaeointensity data from the cryogenian and their potential implications for inner core nucleation age. Geophysical Journal International 226: 66-77, doi: 10.1093/gji/ggab090
Macdonald FA, Wordsworth R (2017) Initiation of Snowball Earth with volcanic sulfur aerosol emissions. Geophysical Research Letters 44: 1938-1946, doi: 10.1002/2016GL072335
Macdonald FA, McClelland WC, Schrag DP, Macdonald WP (2009) Neoproterozoic glaciation on a carbonate platform margin in Arctic Alaska and the origin of the North Slope subterrane. Geological Society of America Bulletin 121: 448-473, doi: 10.1130/B26401.1
Macdonald FA and 8 coauthors (2018) Cryogenian of Yukon. Precambrian Research 319: 114-143, doi: 10.1016/j. precamres.2017.08.015
Macdonald FA and 9 coauthors (2010) Calibrating the Cryogenian. Science 327: 1241-1243, doi: 10.1126/science.1183325
MacLennan SA and 7 coauthors (2020) Geologic evidence for an icehouse Earth before the Sturtian global glaciation. Science Advances 6: eaay6647, doi: 10.1126/sciadv.aay6647
MacLennan SA and 9 coauthors (2018) The arc of the Snowball: U–Pb dates constrain the Islay anomaly and the initiation of the Sturtian glaciation. Geology 46: 539-542, doi: 10.1130/G40171.1
Marshall H, Walker JC, Kuhn WR (1988) Long-term climate change and the geochemical cycle of carbon. Journal of Geophysical Research 93: 791-801, doi: 10.1029/jd093id01p00791
Park Y, Swanson-Hysell N, Macdonald F, Lisiecki L (2020a) Evaluating the relationship between the area and latitude of large igneous provinces and Earth’s long-term climate state. In: Ernst RE, Dickson AJ, Bekker A (eds) Large Igneous Provinces: A Driver of Global Environmental and Biotic Changes, Volume 255 (First Edition). American Geophysical Union, John Wiley and Sons Inc, Washington DC, pp 153-168, doi: 10.1002/9781119507444.ch7
Park Y and 5 coauthors (2020b) Emergence of the Southeast Asian islands as a driver for Neogene cooling. Proceedings of the National Academy of Sciences 117: 2531925326, doi: 10.1073/pnas.2011033117
Park Y and 9 coauthors (2020c) The lead-up to the Sturtian Snowball Earth: Neoproterozoic chemostratigraphy timecalibrated by the Tambien Group of Ethiopia. Geological Society of America Bulletin 132: 1119-1149, doi: 10.1130/ B35178.1
Pierrehumbert R, Abbot D, Voigt A, Koll D (2011) Climate of the Neoproterozoic. Annual Review of Earth and Planetary Sciences 39: 417-460, doi: 10.1146/ annurev-earth-040809-152447
Pu JP and 9 coauthors (2022) Emplacement of the Franklin large igneous province and initiation of Snowball Earth. Science Advances 8: eadc9430, doi: 10.1126/sciadv. adc9430
Rainbird RH (1993) The sedimentary record of mantle plume uplift preceding eruption of the Neoproterozoic Natkusiak flood basalt. The Journal of Geology 101: 305-318
Schaller MF, Wright JD, Kent DV, Olsen PE (2012) Rapid emplacement of the Central Atlantic Magmatic Province as a net sink for CO2. Earth and Planetary Science Letters 323-324: 27-39, doi: 10.1016/j. epsl.2011.12.028
Walker JCG, Hays PB, Kasting JF (1981) A negative feedback mechanism for the long-term stabilization of Earth’s surface temperature. Geophysical Research Letters 86: 9776-9782, doi: 10.1029/ JC086iC10p09776
Williamson N, Ootes L, Rainbird RH, Bedard JH, Cousens B (2016) Initiation and early evolution of the Franklin magmatic event preserved in the 720 Ma Natkusiak Formation, Victoria Island, Canadian Arctic. Bulletin of Volcanology 78: 19, doi: 10.1007/s00445-016-1012-9