October 2023 Issue Table of Contents
Evolution has not been a simple path. Since the first appearance of complex life, there have been several mass extinctions on Earth. This was exemplified by the most severe event during the Phanerozoic, the end-Permian mass extinction that occurred 252 million years ago and saw a loss of 90% and 70% of all marine and terrestrial species, respectively. Such mass extinctions have entirely reset ecosystems. Increasing evidence points to the massive eruption and crustal emplacement of magmas associated with large igneous provinces (LIPs) as key drivers of these events. Understanding how LIP events disrupted global biogeochemical cycles is of prime importance, especially as humans alter the atmosphere and biosphere today. We explore the cascading impacts of LIP events on global climate, oceans, and land—including runaway greenhouses, the release of toxic metals to the environment, the destruction of the ozone layer, and how global oceans are driven to anoxic and acidic states— all of which have parallels in the consequences of modern industrialisation.
1811-5209/23/0019-0276$2.50 DOI: 10.2138/gselements.19.5.276
Keywords: Large igneous province; carbon cycle; mass extinction; global warming; marine anoxia.
INTRODUCTION
Volcanoes have been an ever-present part of Earth history, formed through melting of rock related to subduction, crustal extension, and mantle upwelling (hotspots). Estimates suggest there are ~1350 active volcanoes today, with about 50 of these erupting in any given year. Eruptive episodes range from lava flows seen in Hawaii and Iceland, to more spectacular explosive eruptions such as Mount Pinatubo in the Philippines in 1991 and Hunga TongaHunga Ha’apai in Tonga in 2022. Volumes of material released by volcanoes range from effusive flows of lava < 104 m3, like those in Hawaii, to the ‘mega-colossal’ Oruanui eruption of the Taupō Volcano, New Zealand, that released over 1000 km3 of pyroclastic material around 26,500 years ago. While such eruptions have had global impact on Earth’s ecosystems, and more recently human societies, they differ greatly in both nature and scale to large igneous provinces (LIPs). LIPs are comprised of >100,000 km3 emplacements (injections) of magma into the crust along with associated surface eruptions (Ernst et al. 2021). The largest LIPs involve several million km3 of material, for instance the 122-Ma Ontong-Java Plateau is over 8 million km3 (Ernst et al. 2021). LIPs typically occur as intrusions into the crust and are preserved in the rock record as extensive networks of sills and dykes. Where extruded, submarine or subaerial eruptions are referred to as ‘flood basalts’, as they have, in some cases, truly flooded millions of square kilometres of the Earth’s surface with lava. The Siberian Traps (Fig. 1) cover an area almost the size of Europe, while the Deccan Traps span most of the Indian subcontinent. Fortunately for us, such events have been rare through Earth history. However, understanding how such massive magmatic events have impacted Earth systems and altered the evolutionary pathway throughout the Phanerozoic remains an area of active research. There is growing consensus that global warming and environmental devastation caused by LIP events had dramatic impacts on terrestrial and marine ecosystems. We now know that several of the greatest extinctions in Earth history are temporally linked to LIP events (Bond and Grasby 2017).
HOW LARGE IGENOUS PROVINCES IMPACT GLOBAL ECOSYSTEMS
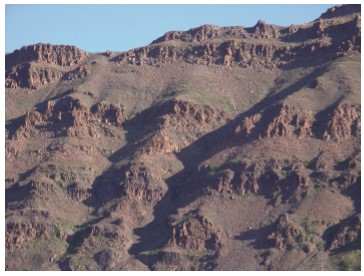
To understand the impact of LIPs, we can search for clues in the local to regional to global ecological impacts of volcanoes. Heavy ash loads, lahars, and lava flows can have devastating effects on the local environment, whereas ejecta comprised of a diverse range of fine solids, gases, and volatiles can have a much wider distribution. Such materials can persist in the atmosphere from minutes to centuries, or even longer in some cases (e.g, carbon dioxide). The atmospheric residence time and dispersal of volcanic ejecta is influenced by particle size, atmospheric reaction rates, and sorption to other particles, along with the eruption intensity, duration, and location. Large explosive eruptions in recent history have driven major changes to weather patterns, crop failure, mass human migration, and even the collapse of societies. Within weeks, the ejecta from the 1991 Mount Pinatubo eruption was distributed globally, cooling global weather for a short time by modifying the sulfate aerosol content of the atmosphere (sulfate aerosols have a strong scattering effect on solar radiation increasing planetary albedo—this has even been proposed as a climate change mitigation strategy). Fissure eruptions at Laki, Iceland (1783–1784) are a better analogue for a LIP event. Laki erupted 15 km3 of basalt (Deegan et al. 2023 this issue), as well as substantial amounts of hydrofluoric acid and sulfur dioxide, that decimated Iceland’s livestock and crops, leading to a famine that killed approximately a quarter of the island’s human population. The estimated 120 million tonnes of sulfur dioxide released by Laki cooled the Northern Hemisphere so much that crops failed and livestock across Europe was poisoned, leading to—according to some historians—the French Revolution. Likewise, the fall of successive Chinese dynasties over the past two millennia has been linked to volcanically induced climate change (Gao et al. 2021). Although such eruptions have been catastrophic from a human perspective, these historical examples are truly insignificant on geological timescales. Earth’s LIPs have intruded and erupted volumes of material several orders of magnitude larger than historical and contemporary volcanic eruptions (millions instead of tens, or very rarely hundreds to thousands, of km3; Ernst et al. 2021).
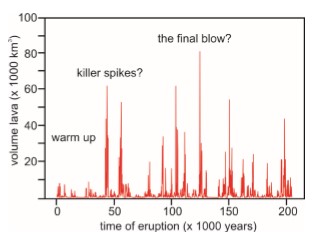
Comparisons between LIPs and historical eruptions are not easily made because many outstanding questions remain concerning how LIPs form—and none have been emplaced since the Columbia River Basalts that formed in the western U.S. 17–14 million years ago (Ernst et al. 2021). A LIP is not a single dramatic event like Mount Pinatubo, or the explosive Mount Tambora eruption in 1815 that released over 150 km3 of material in a matter of hours. U-Pb dating of zircon crystals indicates that LIPs extruded lava flows over tens if not hundreds of thousands of years. For example, the total eruption period of the Deccan Traps in India, closely timed with the Cretaceous–Paleogene extinction (previously known as Cretaceous–Tertiary or K/T extinction) that saw the demise of the dinosaurs, occurred over four ~100,000-year periods of high eruption activity between 66.3 and 65.5 million years ago (Schoene et al. 2019). As such, LIP events are better thought of as a long series of many thousands of seemingly mild Laki-like eruptions, rather than a single massive explosive eruption (Fig. 2). Because of this, timing and tempo are everything when it comes to the destructive power of a LIP. As a thought experiment, we can take the estimated 4 million km3 total volume of the Siberian Traps LIP in Russia that is widely considered to be the driver of Earth’s greatest mass extinction at the end of the Permian period. If the total volume of lava is distributed over a period of 500 ky, this gives an average eruption rate of 8 km3/y. While this result is not significantly higher than what was released by the Laki eruption, such constant and highly productive magmatism is an unlikely modern eruption scenario. Instead, LIP eruptions are a stochastic process, with lava extrusion occurring as a series of sequential eruptions that can be modeled as a multifractal time series (Grasby et al. 2020). Some individual eruptions may not be significant on their own, but at high enough frequency, they could build atmospheric contaminants at rates faster than they are removed, generating increasing stress on biological systems. This itself could be enough to drive a mass extinction, or it could weaken the normal buffering capacity of organisms, making ecosystems more vulnerable to rarer, more extreme eruptions. Earth’s greatest biotic crisis, the Permian–Triassic mass extinction (PTME), is the perfect case study for this. Mounting evidence shows that the early onset of the contemporaneous Siberian Traps LIP eruptions caused increasing stress to global biogeochemical cycles in the lead up to the main extinction event (Dal Corso et al. 2022). This interplay between atmospheric residence time and eruption frequency also influences the relative impacts of global cooling versus global warming caused by different LIP gases, as discussed below.
Beyond the tempo of eruption, location is also a critical factor governing lethality. Given that a LIP could be emplaced anywhere on the planet, a submarine event is most likely, as oceans cover 70% of the planet. Ocean water attenuates the movement of eruptive material because seawater mixing is relatively slow (Percival et al. 2018). A submarine LIP is also less likely to impact terrestrial ecosystems—if emissions do not breach the ocean surface. In contrast, emissions from a subaerial LIP can be quickly spread around the globe with their products deposited on land and ocean, as well as affecting atmospheric chemistry and solar radiation. The type of crust a LIP erupts through also matters. Basalts that form the ocean crust have little reactive material. In comparison, thick sedimentary basins rich in organic matter and evaporite deposits (various salts precipitated from marine water) could be thermally altered by intruding magma and produce significant additional volatile gases, in addition to those generated by the LIP itself. Escape of these gases to the surface may have been a critical factor in what made the PTME the most severe extinction of the Phanerozoic, as discussed below.
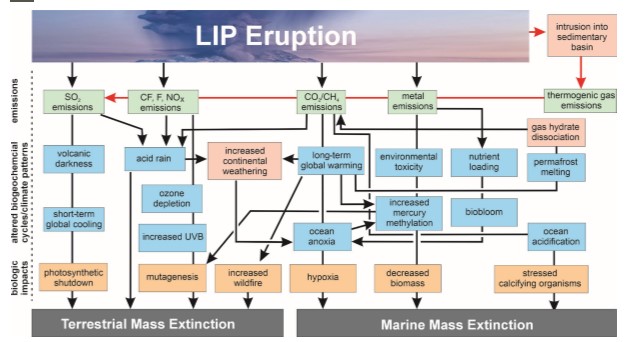
Do LIP Events Heat or Freeze the Earth?
The two volumetrically most important gases released during LIP events are sulfur dioxide (SO2) and carbon dioxide (CO2) (Fig. 3). Sulfur dioxide emissions have a short-term warming effect that lasts days to weeks. Over slightly longer timescales, SO2 reacts with particles in the atmosphere to form sunlight-blocking sulfate aerosols that cause cooling for 2–3 years after an eruption, before being finally depleted from the atmosphere through rain out (notably, that rain is acid—given that 2SO2 + O2 + 2H2O = 2H2SO4, i.e., sulfuric acid). Therefore, despite the short-term warming, SO2 emissions have a net cooling effect on the planet. As discussed above, the cooling effect of volcanoes is well documented in the aftermath of some of the largest eruptions in human history, such as ‘the year without summer’ in the Northern Hemisphere following the 1815 Tambora eruption. Such impacts are not scalable to a LIP. The conversion of SO2 to sun-blocking sulfate aerosols is limited by the amount of oxidants in the atmosphere, and sulfate aerosols also tend to increase in size with increasing SO2 injection, limiting their optical depths and atmosphere residence time (Schmidt et al. 2016). Given these self-limiting processes, LIP eruptions injecting more SO2 into the atmosphere do not necessarily drive greater cooling. Modeling suggests that cooling is short lived, as sulfate aerosols get quickly washed out from the atmosphere, and could only drive a death-by-cold extinction if there were regular, sustained eruptions during a LIP event (Schmidt et al. 2016). Convincing evidence for devastating volcanic winter scenarios associated with LIP events is lacking, although this scenario has been invoked for the Late Ordovician mass extinction (LOME) ~443 million years ago (Brenchley et al. 2001)—the second largest extinction of the Phanerozoic. LIP events instead appear to be associated with geologically rapid, and in some cases extreme, global warming (Sun et al. 2012).
As a major gaseous product of magmatism, CO2 has an important influence on global climate, driving warming in a manner similar to modern day anthropogenic emissions. A key difference between SO2 and CO2 is that the atmospheric residence time of the latter (thousands of years) is much longer than that of the former (tens of years). The longer atmospheric residence time of CO2 offsets the cooling effects of short-term-residence SO2, but, again, it is the release rate rather than the total mass that controls the environmental and ecological impacts of eruptions, because normal weathering processes are enough to draw down most volcanogenic CO2. Theoretically at least, global warming associated with CO2 emissions increases the rates of chemical weathering, accelerating CO2 drawdown in a positive feedback loop.
When it comes to LIPs, the impact of emissions on climate was very likely significant, although it has been questioned whether volcanic eruptions alone were capable of driving the climate warming associated with extinction events. Single volcanic eruptions are unlikely to produce large enough CO2 emissions to drive calamitous climatic effects. However, it is estimated that the Siberian Traps released up to 30,000 Gt CO2 (ten times the mass in today’s atmosphere). This figure increases significantly when accounting for CO2 released from contact metamorphism of organic-rich sediments. Thermogenic gases emitted when LIPs intruded into sedimentary basins rich in organic matter (coal, petroleum, organic-rich shales), which were effectively coked along the margins of magma intrusions or combusted in near-surface environments, are a key component of many extinction scenarios. This process may provide the ‘missing link’ in the PTME (Svensen et al. 2018), whereby the Siberian Traps LIP erupted through the Tunguska Basin of Russia, generating additional CO2 and methane (CH4) from the burning of organic-rich sediments. This hypothesis has gained traction in studies addressing the PTME and the Triassic–Jurassic extinction in recent years. Significant organic combustion at the PTME is supported by the discovery of ash particles, remarkably similar to those produced by modern coal-burning power plants, in sediments deposited across the PTME around 20,000 km downwind of the Siberian Traps. Evidence of melted and combusted organics within the solidified lavas of the Siberian Traps themselves lends further support for a causal role of thermogenic greenhouse gas emissions (Elkins-Tanton et al. 2020). Abundant diatreme pipes that date to the time of the Siberian Traps eruptions in the Tunguska Basin (Polozov et al. 2016) are thought to be the very escape structures formed when gases produced in the subsurface became overpressured and blasted their way upwards through the overlying bedrock before entering the atmosphere.
Additional greenhouse gas sources with indirect origins in LIP events include gas hydrates. These are deposits of methane, a significantly more effective greenhouse gas than CO2, that are trapped in frozen ground at high latitudes and on the ocean floor. It was suggested that CO2-driven global warming could reach a tipping point at which the melting of methane hydrates drives further warming in a runaway greenhouse scenario (Dickens et al. 1995). However, gas hydrate melting is an endothermic reaction and, thus, a rate-limiting process that may make the emission rates too slow (Majorowicz et al. 2014) to drive a runaway greenhouse scenario.
Ocean Anoxia and Acidification
Increased atmospheric CO2 resulting from a LIP eruption has significant impact beyond climate warming and the associated thermal stress placed on organisms (Fig. 3). Increased seawater temperature reduces its capacity to dissolve oxygen, while increased partial pressure of atmospheric CO2 increases its dissolution into seawater, driving a decrease in ocean pH by formation of carbonic acid (CO2 + H2O + CO3−2 ↔ 2HCO3−). Oceanic anoxic events are observed throughout the Phanerozoic in association with large LIP eruptions. These can range from periods of expanded oxygen minimum zones to periods where biomarker evidence suggests that euxinic conditions (dissolved hydrogen sulfide in the water) extended into the photic zone, both of which greatly reduce the habitable areas for marine life. When oxygen minimum zones expand onto marine shelves, where much complex life resides, the oceans quickly become wastelands suitable for only microbial life, which can then thrive and grow into extensive mats when not being grazed upon. The expansion of euxinic conditions is highly toxic for most higher life forms and creates ocean conditions akin to those of Precambrian oceans, prior to oxygenation of the oceans. Indeed, in the aftermath of the PTME, the rock record has several features in common with the Precambrian, such as the prevalence of stromatolites (fossilised microbial mats that form in the absence of higher life forms), as if Earth had wound the clock back 500 million years. However, the survival and subsequent radiation of life following mass extinctions indicates that, even in the worst conditions, isolated ‘habitable zones’ must have served as refugia from which complex life was able to expand—once suitable conditions returned.
Models also suggest that large inputs of LIP CO2 into the atmosphere could cause the carbonate saturation horizon (a function of ocean pH) to shoal by 1 km due to increased acidity (Huybers and Langmuir 2009). Shoaling would narrow the depth in the ocean where carbonates are thermodynamically favoured, thus placing increased stress on carbonate secreting organisms by making it more energy intensive to grow and maintain their skeletons and protective shells. There exist evolutionary adaptions to low-pH environments such as growth of protective membrane coatings, smaller body sizes, reduced shell ornamentations, and reduced surface area relative to body size. The boron isotope composition of marine carbonates (δ11B) is a recently developed proxy used to measure ancient ocean pH. Across the PTME extinction boundary, δ11B values show a positive shift, consistent with a fall in pH in response to the Siberian Traps LIP (Jurikova et al. 2020).
Evidence for lysocline shoaling (the ocean depth below which carbonate dissolution is enhanced) in response to falling pH is also observed across northwestern Pangea at this time (Beauchamp and Grasby 2012). Here, carbonate producers, whose shells dissolve under low-pH conditions, were eradicated from deep to shallow water environments and replaced by acid-resistant siliceous sponges that normally only inhabit the deep sea. At the same time, carbonate producers in the Tethys Ocean show evidence for decreased body size and loss of energetically intensive ornamentation.
Ozone-Destroying Halogens and Acid Rain
Other environmentally important gases released during LIP events include halogen gases, such as chlorine and fluorine, that deplete atmospheric ozone (e.g., Cl + O3 → ClO + O2 and then ClO + O → Cl + O2 to restart the cycle). In the 1970s and 80s, there was major concern that chlorofluorocarbons (CFCs) were depleting stratospheric ozone that absorbs much of the sun’s ultraviolet B (UV-B) rays, and LIPs can scale this problem up dramatically. Ozone depletion leads to deadly enhanced UV-B radiation on land and in shallow marine settings. Above we discussed how a LIP intruded into a sedimentary basin can lead to thermal metamorphism of organic matter, generating additional thermogenic greenhouse gases. Sedimentary basins can also host thick deposits of evaporites, which include layers of halite (i.e., salt, NaCl) and anhydrite (CaSO4) formed through the evaporation of seawater. Thermal metamorphism of such deposits can generate SO2 along with halocarbons like methyl chloride and methyl bromide, all of which can cause significant stratospheric ozone depletion. While a collapse of the ozone layer and consequent exposure of terrestrial flora to high levels of solar UV-B radiation could be consistent with findings of mutated spores and pollen associated with the PTME and the Triassic–Jurassic extinction, these may also be the result of the toxicity of mercury and other metals released by LIPs (Lindström et al. 2019; Chu et al. 2021). Elevated UV-B–absorbing compounds in the outer wall of sporomorphs (produced by plants to protect against UV-B radiation) are known from the levels of mercury spikes (as discussed in the next section) and a major negative carbon isotope excursion (also a LIP fingerprint), strengthening the link between LIP events and terrestrial extinctions (Liu et al., 2022).
Along with causing ozone depletion, release of SO2 also forms sulfuric acid by reaction with moisture in the atmosphere (2SO2 + O2 → 2SO3 + H2O → H2SO4). Modeling has suggested that volumes of sulfuric acid released by the Siberian Traps could have been sufficient to affect terrestrial ecosystems in the Late Permian. Indeed, vanillian compounds discovered at PTME boundary sections in northern Italy support severe soil acidification (Sephton et al. 2015), further linking terrestrial extinctions to a LIP event.
Toxic Metals
LIP events are also a significant source of numerous metal emissions to the atmosphere, including many that are highly toxic to higher life forms, and some such as mercury (Hg) that are deadly across the entire ecosystem. Other released metals such as cadmium (Cd) and nickel (Ni) have more cryptic relationships with the biosphere, acting as essential nutrients at certain trophic levels, but serving as deadly toxins for others. Evidence hints at large metal releases during LIP events, in the form of significant spikes in concentrations in sedimentary records. Most notable are global Hg spikes observed at several mass extinction events. Models suggest that these Hg spikes are not just signatures of a LIP event in the sedimentary record, but that they could also reflect the build-up of toxic levels of Hg in the global ecosystem (Grasby et al. 2020). If correct, coating the planet with a pulse of toxic metals is one potential explanation for the closely spaced occurrence of both terrestrial and marine extinctions.
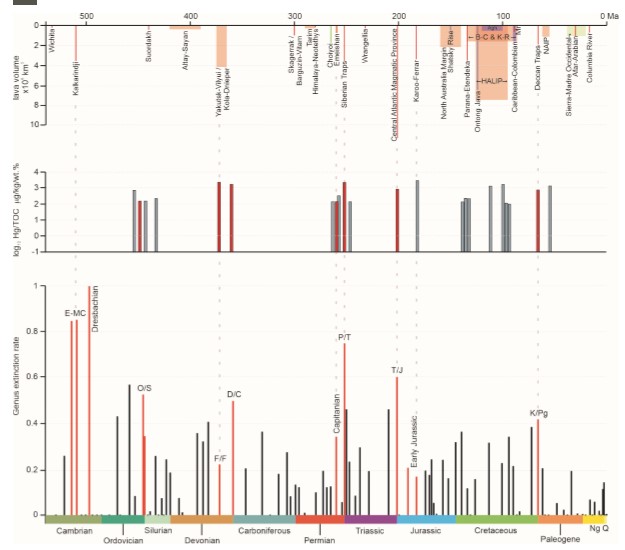
DEATH BLOWS OF THE PHANEROZOIC
LIPs events were implicated as a driver of mass extinctions as early as the 1970s (Kennett and Watkins 1970). In the following decades, the challenge was to definitively link the age of a mass extinction, often only biostratigraphically controlled, with the timing of a specific LIP event (Fig. 3), for which variable quality radio-isotopic dating was available. Greatly improved dating techniques (see Ernst et al. 2021 and references within; Gaynor et al. 2023 this issue), along with geochemical stratigraphic tools such as mercury spikes in sediments, have uncovered a temporal link between LIP events and four of the ‘Big Five’ extinction events of the Phanerozoic (Fig. 4), as well as every minor crisis since the Permian (Bond and Grasby 2017; Grasby et al. 2019). In some cases, the LIP-extinction driver remains contested as is the case of the LOME, where the link is somewhat indirect (Gong et al. 2017; Bond and Grasby 2020). Here, there are several lines of evidence in the sedimentary record (e.g., rapid warming, mercury spikes, carbon isotope anomalies) that are suspiciously similar to those observed at other LIP-caused mass extinctions, yet there is no clear LIP in the geologic record at that time. This could be explained by the poor age control on several candidate LIPs or simple erosion or subduction of the LIP deposits themselves. Elsewhere in time, at the Cretaceous/ Paleogene boundary, understanding of the extinction is complicated by the contemporaneous impact of a large bolide (Alvarez et al. 1980). That crisis coincided with one of the larger LIP events of the Phanerozoic (the ~1 million km3 of the Deccan Traps of India) and consequently the extinction scenario remains one of the most hotly debated (Burgess 2019).
CONCLUSIONS
Several decades of research effort have generated sufficient evidence for a close temporal link between LIP events and multiple mass extinctions. While this does not prove causation, the frequency of the link suggests that it is more than a coincidence. However, many LIPs are not associated with significant extinction losses, especially the giant oceanic plateaus of the Cretaceous that appear to have driven oceanic anoxic events without mass extinctions (e.g., Percival et al. 2018). Clearly, not all LIPs are deadly, and factors controlling their lethality remain debated. These may include continental configuration, subaerial versus submarine eruptions, and whether they intruded into sedimentary basins rich in organics and evaporites. A key lesson from the deep time record is that large emissions of CO2 have widespread and deadly impacts—on a global scale. Once again, rate is everything. The current emissions of 37 Gt CO2 added to the atmosphere every year would, within 800 years, replicate the estimated 30,000 Gt of CO2 released by the Siberian Traps—perhaps too quickly for normal buffering systems to keep up. Will the modern phase of atmospheric change be a killer event like some LIPs of the past? We are starting to learn about what makes a LIP deadly, but a truly meaningful evaluation of the impacts of carbon emissions on global ecosystems requires continued studies of both modern and deep-time Earth systems.
ACKNOWLEDGMENTS
DPGB acknowledges NERC Advanced Research Fellowship (grant number NE/J01799X/1), which supported his time whilst researching the links between volcanism and extinction.
REFERENCES
Alvarez LW, Alvarez W, Asaro F, Michel HV (1980) Extraterrestrial cause for the Cretaceous-Tertiary extinction. Science 208: 1095-1108, doi: 10.1126/ science.208.4448.1095
Beauchamp B, Grasby SE (2012) Permian lysocline shoaling and ocean acidification along NW Pangea led to carbonate eradication and chert expansion. Palaeogeography, Palaeoclimatology, Palaeoecology 350-352: 73-90, doi: 10.1016/j.palaeo.2012.06.014
Bond DPG, Grasby SE (2017) On the causes of mass extinctions. Palaeogeography, Palaeoclimatology, Palaeoecology 478: 3-29, doi: 10.1016/j.palaeo.2016.11.005
Bond DPG, Grasby SE (2020) Late Ordovician mass extinction caused by volcanism, warming, and anoxia, not cooling and glaciation. Geology 48: 777-781, doi: 10.1130/G47377.1
Brenchley PJ, Marshall JD, Underwood CJ (2001) Do all mass extinctions represent an ecological crisis? Evidence from the Late Ordovician. Geological Journal 36: 329-340, doi: 10.1002/gj.880
Burgess S (2019) Deciphering mass extinction triggers. Science 363: 815-816, doi: 10.1126/science.aaw0473
Chu D and 9 coauthors (2021) Metal-induced stress in survivor plants following the end-Permian collapse of land ecosystems. Geology 49: 657-661, doi: 10.1130/ G48333.1
Dal Corso J and 8 coauthors (2022) Environmental crises at the Permian– Triassic mass extinction. Nature Reviews Earth & Environment 3: 197-214, doi: 10.1038/s43017-021-00259-4
Deegan FM, Callegara S, Davies HFL, Svensen HH (2023) Driving global change one LIP at a time. Elements 19: 269-275
Dickens GR, O’Neil JR, Rea DK, Owen RM (1995) Dissociation of oceanic methane hydrate as a cause of the carbon isotope excursion at the end of the Paleocene. Paleoceanography 10: 965-971, doi: 10.1029/95PA02087
Elkins-Tanton LT and 5 coauthors (2020) Field evidence for coal combustion links the 252 Ma Siberian Traps with global carbon disruption. Geology 48: 986-991, doi: 10.1130/G47365.1
Ernst RE and 8 coauthors (2021) Large igneous province record through time and implications for secular environmental changes and geological time-scale boundaries. In: Ernst RE, Dickson AJ, Bekker A (eds) Large Igneous Provinces: A Driver of Global Environmental and Biotic Changes, Volume 255 (First Edition). American Geophysical Union, John Wiley and Sons Inc, Washington DC, pp 3-26, doi: 10.1002/9781119507444.ch1
Gao C and 8 coauthors (2021) Volcanic climate impacts can act as ultimate and proximate causes of Chinese dynastic collapse. Communications Earth & Environment 2: 234, doi: 10.1038/ s43247-021-00284-7
Gaynor SP, Davies HLF, Schaltegger (2023) High-precision geochronology of LIP intrusions: records of magma–sediment interaction. Elements 19: 302-308
Gong Q and 8 coauthors (2017) Mercury spikes suggest volcanic driver of the Ordovician-Silurian mass extinction. Scientific Reports 7: 5304, doi: 10.1038/ s41598-017-05524-5
Grasby SE, Them TR, Chen Z, Yin R, Ardakani OH (2019) Mercury as a proxy for volcanic emissions in the geologic record. Earth-Science Reviews 196: 102880, doi: 10.1016/j.earscirev.2019.102880
Grasby SE, Liu X, Yin R, Ernst RE, Chen Z (2020) Toxic mercury pulses into late Permian terrestrial and marine environments. Geology 48: 830-833, doi: 10.1130/ G47295.1
Green T, Renne PR, Keller CB (2022) Continental flood basalts drive Phanerozoic extinctions. Proceedings of the National Academy of Sciences 119: e2120441119, doi: 10.1073/ pnas.2120441119
Huybers P, Langmuir C (2009) Feedback between deglaciation, volcanism, and atmospheric CO2. Earth and Planetary Science Letters 286: 479-491, doi: 10.1016/j.epsl.2009.07.014
Jurikova H and 10 coauthors (2020) Permian–Triassic mass extinction pulses driven by major marine carbon cycle perturbations. Nature Geoscience 13: 745-750, doi: 10.1038/s41561-020-00646-4
Kennett JP, Watkins ND (1970) Geomagnetic polarity change, volcanic maxima and faunal extinction in the South Pacific. Nature 227: 930-934, doi: 10.1038/227930a0
Lindström S and 8 coauthors (2019) Volcanic mercury and mutagenesis in land plants during the end-Triassic mass extinction. Science Advances 5: eaaw4018, doi: 10.1126/sciadv.aaw4018
Liu F and 7 coauthors (2023) Dying in the sun: direct evidence for elevated UV-B radiation at the end-Permian mass extinction. Science Advances 9: eabo6102, doi: 10.1126/sciadv.abo6102
Majorowicz J, Grasby SE, Safanda J, Beauchamp B (2014) Gas hydrate contribution to Late Permian global warming. Earth and Planetary Science Letters 393: 243-253, doi: 10.1016/j.epsl.2014.03.003
Muscente AD and 6 coauthors (2022) Appearance and disappearance rates of Phanerozoic marine animal paleocommunities. Geology 50: 341-345, doi: 10.1130/ G49371.1
Percival LME and 10 coauthors (2018) Does large igneous province volcanism always perturb the mercury cycle? Comparing the records of Oceanic Anoxic Event 2 and the end-Cretaceous to other Mesozoic events. American Journal of Science 318: 799-860, doi: 10.2475/08.2018.01
Polozov AG and 5 coauthors (2016) The basalt pipes of the Tunguska Basin (Siberia, Russia): high temperature processes and volatile degassing into the end-Permian atmosphere. Palaeogeography, Palaeoclimatology, Palaeoecology 441: 51-64, doi: 10.1016/j.palaeo.2015.06.035
Schmidt A and 11 coauthors (2016) Selective environmental stress from sulphur emitted by continental flood basalt eruptions. Nature Geoscience 9: 77-82, doi: 10.1038/ ngeo2588
Schoene B and 6 coauthors (2019) U-Pb constraints on pulsed eruption of the Deccan Traps across the end-Cretaceous mass extinction. Science 363: 862-866, doi: 10.1126/science.aau2422
Sephton MA, Jiao D, Engel MH, Looy CV, Visscher H (2015) Terrestrial acidification during the end-Permian biosphere crisis? Geology 43: 159-162, doi: 10.1130/ G36227.1
Sun Y and 7 coauthors (2012) Lethally hot temperatures during the Early Triassic greenhouse. Science 338: 366-370, doi: 10.1126/science.1224126
Svensen HH and 8 coauthors (2018) Sills and gas generation in the Siberian Traps. Philosophical Transactions of the Royal Society A 376: 20170080, doi: 10.1098/ rsta.2017.0080